Reviews
in Undergraduate Research - Issue 1
The
Role of Caveolae and the Caveolins in Mammalian Physiology
}
Dr. Babak Razani and Dr. Michael Lisanti
Albert Einstein College of Medicine
Dept. of Molecular Pharmacology
|
SUMMARY
Caveolae are 50-100 nm invaginations of the plasma membrane that have
captured the interest of scientists for many decades. However, the wide-ranging
and physiologically important roles of these curious structures have
only recently been addressed. Among the important milestones in the
understanding of caveolae is the discovery of a family of proteins that
are intimately involved in caveolar function (the caveolins). It has
become clear now that caveolae and their caveolin "marker proteins"
are involved in a variety of cellular processes including endocytosis,
lipid homeostasis, signal transduction, and tumorigenesis. In this review,
we will highlight the current view of caveolae in cell biology and discuss
the relevance of these structures to mammalian physiology.
INTRODUCTION TO
CAVEOLAE
Examination of a cell at the ultrastructural level reveals numerous
intricate components that contribute to its appropriate function. Since
the advent of electron microscopy in the 1940s and 50s, structures such
as the mitochondrion, endoplasmic reticulum, golgi apparatus, and clathrin-coated
endocytic vesicles were discovered for the first time and their distinct
functions in cells speculated upon. In this same period, another cellular
entity, a 50-100 nm vesicle that was found to be either directly invaginated
from or in close proximity to the plasma membrane was also described
(Figure 1A). Based on this conspicuous "cave-like" morphology
at the membrane, these structures were named "caveolae"
and were added to the growing list of newly discovered cellular organelles
(Palade, 1953; Yamada, 1955).
In the ensuing decades and with the incipience of cellular and molecular
biology, research on many of these cellular organelles led to a precise
understanding of their function (e.g. implication of mitochondria in
ATP production, the ER/golgi in protein synthesis and sorting, and clathrin-coated
pits in endocytosis). Unfortunately, due to difficulty in characterizing
their biochemical and molecular nature, caveolae remained enigmatic
structures with no definitive function(s). Based on their structural
resemblance to clathrin-coated vesicles and their seemingly dynamic
movement between the plasma membrane and intracellular compartments,
caveolae were initially thought to serve solely an endocytic role akin
to clathrin-coated pits (Palade, 1953; Simionescu et al., 1975).
Now, based on work in the last decade, caveolae are being recognized
as rather complex organelles with important roles not only in endocytosis
but also lipid homeostasis, signal transduction, and tumorigenesis.
In addition, they seem to play very specific roles in distinct cell
types, making these structures one of the most interesting and multi-functional
entities in cells. In this review, we will discuss the salient features
of these structures and the current understanding of their function
in mammalian organisms.
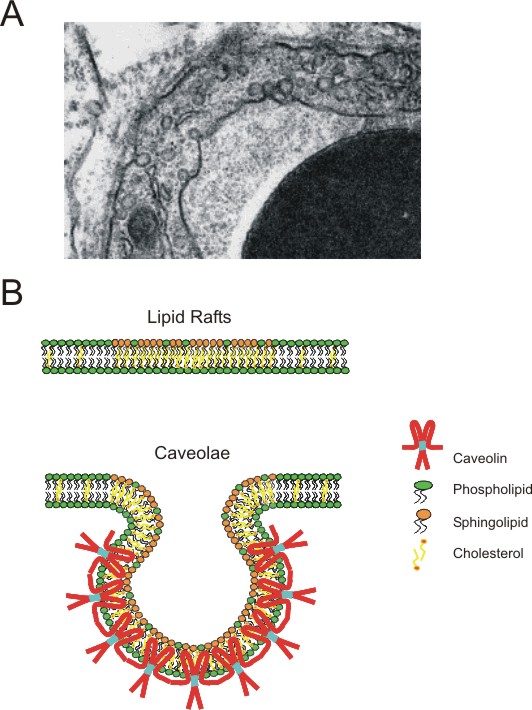 |
Figure
1. Caveolae, a unique "cellular organelle" with a unique
"marker protein"
A)
Electron micrograph of an endothelial cell showing caveolae, 50-100
nm structures that are either direct invaginations or in close proximity
to the plasma membrane. Caveolae are estimated to make-up an estimated
30-70% of the plasma membrane area in certain cells such as endothelial
cells, adipocytes, or Type I pneumocytes .
B) Diagram comparing the biochemical composition of lipid
rafts and caveolae (adapted from (Galbiati et al., 2001)). Lipid
rafts form via a coalescence of cholesterol and sphingolipids; as
a result, these microdomains have vastly different biochemical properties
than the bulk phospholipids bilayer. Caveolae are generally considered
to be "invaginated" lipid rafts primarily due to an enrichment
in a family or proteins known as the caveolins. Here, the caveolin
oligomer is depicted as a dimer for simplicity. |
The biochemical/structural
nature of caveolae: introduction to the caveolins
Based on numerous biophysical and biochemical analyses of plasma membranes,
it is now known that the traditional view of a lipid bilayer as a
"fluid mosaic" is not entirely accurate (Brown and London,
1998). Although a membrane solely made of phospholipids does indeed
act as a fluid-mosaic, cell membranes which are also composed of cholesterol,
sphingolipids, and various lipid-modified and transmembrane proteins,
behave differently (Brown and London, 1998). In cell membranes, depending
on the local concentration of cholesterol, sphingolipids, and some
phospholipids, more rigid patches of membrane can form. Floating among
the bulk phospholipids bilayer, these biochemically distinct patches
of membrane have now been termed lipid rafts, the study
of which is an active area of research (see (Simons and Toomre, 2000)
for review) (Figure 1B).
Interestingly, research in the past decade has shown that caveolae
are biochemically indistinguishable from lipid rafts and are composed
of a similar local enrichment of cholesterol and sphingolipids (Simons
and Toomre, 2000) (Figure 1B). The primary difference between these
two entities is the invaginated, vesicular morphology of caveolae.
This difference arises due to the presence of a set of proteins unique
to caveolae but absent from lipid rafts, the caveolins.
The caveolin protein family is composed of three distinct proteins,
caveolin-1, -2, and -3 (Cav-1, -2,-3) (Glenney, 1992; Rothberg et
al., 1992; Scherer et al., 1996; Tang et al., 1996). Not surprisingly,
these proteins are expressed in tissues with a high abundance in caveolae;
Cav-1 and -2 are co-expressed in many cell types with especially high
levels in endothelial cells, adipocytes, and type I pneumocytes, while
Cav-3 is exclusively expressed in skeletal and cardiac muscle cells
(Scherer et al., 1994; Scherer et al., 1996; Tang et al., 1996). The
main exception is smooth muscle cells, where intriguingly all three
proteins are expressed (Tang et al., 1996).
The caveolin proteins have several properties which are important
not only for selective localization to caveolae but also for driving
the invagination of these structures. Cav-1 has been shown to have
high binding affinity for cholesterol and sphingolipids (Fra et al.,
1995; Murata et al., 1995; Thiele et al., 2000). This property, along
with three carboxy-terminal lipid-modifications (palmitoylations),
stabilizes and targets Cav-1 to caveolae (Dietzen et al., 1995; Monier
et al., 1996). The caveolins can also oligomerize into a complex of
14-16 subunits and thereafter form even larger mega-complexes by oligomer-oligomer
interactions (Monier et al., 1995; Sargiacomo et al., 1995; Song et
al., 1997). Although it is still largely speculative, it is thought
that the high affinity for cholesterol, the oligomerization, and the
oligomer-oligomer interactions can together form an environment in
the lipid bilayer conducive for the creation of 50-100 nm caveolar
invaginations.
Caveolae, caveolins,
and endocytic processes
The observation that caveolae can exist as invaginations of the plasma
membrane, as completely enclosed vesicles, or as aggregates of several
vesicles, led investigators to infer that these structures were conduits
for the endocytosis of macromolecules (Simionescu et al., 1975). Indeed,
tracer studies and high resolution electron microscopy has revealed
that cells predominantly use caveolae for the selective uptake of
molecules as small as folate to full size proteins such as albumin
and alkaline phosphatase (Anderson et al., 1992; Parton et al., 1994;
Predescu et al., 1997; Schnitzer et al., 1994) (Figure 2A). In endothelial
cells, the uptake of such molecules is complicated by the fact that
the endocytosed caveolae seem to migrate from the luminal side to
the abluminal side, thereby transferring specific serum molecules
to the underlying tissue (a process referred to as transcytosis)
(Predescu et al., 1997; Simionescu et al., 1975) (Figure 2A).
Interestingly, several studies have also shown that caveolae-mediated
uptake of materials is not limited to macromolecules; in certain cell-types,
viruses (e.g. simian virus 40) and even entire bacteria (e.g. specific
strains of E. Coli) are engulfed and transferred to intracellular
compartments in a caveolae-dependant fashion (Anderson et al., 1996;
Montesano et al., 1982; Shin et al., 2000). Although the molecular
mechanism for these endocytic events are not completely understood,
there are indications that the same machinery operating traditional
vesicle budding and fusion processes is functional in this setting
(Henley et al., 1998; Oh et al., 1998; Schnitzer et al., 1995). Thus,
the cell utilizes similar endocytic techniques to differentially traffic
cellular materials.
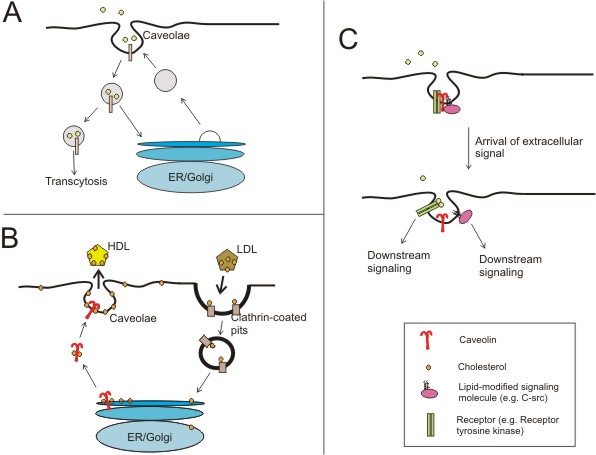 |
Figure
2. Proposed functions of caveolae and the caveolins (adapted from
(Razani and Lisanti, 2001))
A) Certain molecules have been shown to be predominantly
endocytosed via caveolae and not clathrin-coated vesicles. The
fate the cargo in a fully invaginated caveola is not entirely
understood; however, there is evidence to suggest that depending
on the cell type, caveolae can deliver their contents to the ER/golgi
compartments or to the abluminal side of a cell.
B) Intracellular cholesterol is thought to be transported
to plasma membrane caveolae via a golgi-independent caveolin-mediated
route. Caveolae then can serve as "relay stations" to
deliver the membrane cholesterol to the bulk plasma membrane or
to cholesterol-transporters such as HDL particles.
C) Caveolae are now thought to act as signalosomes, or
entities in which signal transduction events can take place efficiently.
A higher level of regulatory complexity is provided by the caveolins
where signaling molecules can be bound until extracellular ligands
relieve them of inhibition. Here, the dynamic regulation of a
receptor tyrosine kinase (e.g. EGF receptor) and a lipid-modified
kinase (e.g. the src-tyrosine kinase) in caveolae are shown. |
Caveolae, caveolins,
and cholesterol homeostasis
Caveolae are highly enriched in cholesterol as compared to the bulk
plasma membrane and Cav-1 binds this cholesterol with high affinity
(estimated at 1 cholesterol molecule per caveolin molecule) (Murata
et al., 1995; Thiele et al., 2000). Furthermore, pharmacological depletion
of plasma membrane cholesterol leads to a loss of morphologically
identifiable caveolae (i.e. "flattening" against the membrane)
and dissipation of the caveolin-matrix (Rothberg et al., 1992). Due
to these observations, it was suggested that caveolae and caveolins
are involved in maintaining intracellular cholesterol balance; indeed,
there is evidence for such a role.
Cellular cholesterol is derived from two main sources, de novo production
or extracellular uptake (via low density lipoprotein (LDL) receptors
localized in clathrin-coated vesicles) (Fielding and Fielding, 1997;
Simons and Ikonen, 2000) (Figure 2B). Once inside, the caveolins seem
to function as intracellular escorts for the transport of this cholesterol
from the endoplasmic reticulum to plasma membrane caveolae (Smart
et al., 1996; Uittenbogaard et al., 1998) (Figure 2B). Upon delivery,
this cholesterol has three fates: (1) to remain as a component of
caveolar cholesterol, aiding in the invagination and proper function
of these structures, (2) to be siphoned into the bulk plasma membrane,
repleting the lipid bilayer with appropriate amounts of cholesterol,
or (3) to be effluxed to serum cholesterol-transporting units like
high density lipoproteins (HDLs) (Fielding et al., 1999; Fielding
and Fielding, 1995; Smart et al., 1996) (Figure 2B). In essence, the
caveolins deliver intracellular cholesterol to a "relay station"
wherein the overall fate of cholesterol is determined; the cholesterol
needs of the cell are met and excesses are effluxed.
Caveolae, caveolins,
and signal transduction
The intimate relationship between caveolae and their protein components,
the caveolins, is obvious. An important question that remained was
whether other plasma membrane proteins can also preferentially localize
to these structures. This issue has been addressed using biochemical
purification, wherein caveolae can be selectively isolated from other
cellular constituents and their protein components analyzed (Lisanti
et al., 1994; Sargiacomo et al., 1993).
Caveolae are highly enriched in numerous membrane-bound proteins,
especially signaling proteins with lipid-modified groups (e.g. H-ras,
src-family tyrosine kinases, heterotrimeric G-proteins, eNOS, etc)
(Lisanti et al., 1994; Smart et al., 1999) (Figure 2C). Furthermore,
it appears that the caveolins are not innocent by-standers in this
environment and can bind and functionally regulate (mostly inhibit)
several of these caveolae-localized molecules (Feron et al., 1996;
Garcia-Cardena et al., 1996; Li et al., 1996; Li et al., 1995; Song
et al., 1996; Song et al., 1997) (Figure 2C). The caveolins possess
a 20 amino acid juxtamembrane domain (now appropriately called the
scaffolding domain) that mediates this functional binding (Okamoto
et al., 1998).
The predilection for signaling proteins to localize to caveolae and
the capacity for the caveolins to regulate their function has led
some to refer to caveolae as "signalosomes" (or bodies where
signal transduction events and cross-talk between different signaling
pathways can take place efficiently and in regulated fashion) (Lisanti
et al., 1994; Smart et al., 1999). This aspect of caveolae is currently
an active area of research since it brings together the interests
of investigators conducting research in seemingly disparate areas.
Caveolae, caveolins,
and tumorigenesis
An interesting corollary to the above-mentioned signalosome concept
arises during tumorigenesis. Several of the proteins localized to
caveolae and inhibited by Cav-1 (namely EGFR, Her2/Neu, and PDGF receptor
tyrosine kinases, components of the Ras/p42/44 MAP kinase cascade,
and members of the PI-3-kinase cascade) ) (Couet et al., 1997; Engelman
et al., 1998; Liu et al., 1996; Yamamoto et al., 1999; Zundel et al.,
2000) are extremely important in pro-proliferative/anti-apoptotic
signaling. If functionally deranged, such proteins can result in cells
with hyperactive cell cycles and eventually tumor formation. In this
regard, caveolae and Cav-1 might be expected to be essential members
of the cellular tumor suppressor repertoire, acting to dampen the
action of tumorigenic signals.
Interestingly, it has been observed that caveolae are absent or reduced
in number and Cav-1 is transcriptionally down-regulated in numerous
cancers (both cell-lines and in situ carcinomas) (Engelman et al.,
1998; Koleske et al., 1995; Lee et al., 1998; Razani et al., 2000).
In addition, both human CAV-1 and -2 genes map to 7q31.1 (a region
of the chromosome found to be frequently deleted in several epithelial
cancers - e.g. breast, lung, renal, and ovary) (Kerr et al., 1996;
Shridhar et al., 1997; Zenklusen et al., 1994). Such observations
provide strong evidence for a caveolin-mediated tumor surveillance
process and give impetus for researchers to include the caveolins
as important factors in the diagnosis and treatment of cancer.
In vivo relevance of
caveolar function in mammalian physiology
The current understanding of caveolae and caveolin function is based
on research conducted either in vitro (biochemical or cell culture
systems) or in vivo (namely, morphological assessment by electron
microscopy). Although such techniques are useful in providing insights
into the functions of these structures, a complete understanding of
their physiological relevance can only be attained by experiments
conducted in the whole organism (e.g. creation of transgenic or knockout
mice, wherein the expression of one or more caveolin proteins is perturbed).
Indeed, in the past year, several groups have reported on the phenotypes
of mice with targeted disruptions of the CAV-1, -2, and -3 loci, thereby
providing the first rigorous assessment of caveolae function in vivo
(Drab et al., 2001; Galbiati et al., 2001; Hagiwara et al., 2000;
Razani et al., 2002; Razani et al., 2001; Razani et al., 2002). Mice
deficient in Cav-1 or Cav-3 (but not Cav-2) lack morphologically identifiable
caveolae in tissues expressing those genes. This observation is important
in that it directly proves the importance of caveolin expression in
caveolae formation and provides a tool for the study of not only caveolins
but caveolae in a mammalian organism.
The phenotypes of Cav-1
null mice (Drab et al., 2001; Razani et al., 2002; Razani et al.,
2001):
- Loss of caveolae in cells
expressing Cav-1 (e.g. endothelial, epithelial, adipose cells)
- Dramatic reduction of
Cav-2 protein levels due to destabilization and degradation via the
proteosomal pathway - thus, Cav-1 null mice are in essence Cav-1 and
-2 deficient
- Histologically abnormal
lungs - thickened alveolar septa due to endothelial cell hyper-proliferation
and increased deposition of extracellular matrix
- Cell cycle defects - fibroblasts
derived from these mice have increased S-phase fractions and proliferate
faster than their wild-type counterparts
- Defects in vasoregulation
- the aortas from these mice are hyper-responsive to vasodilatory
stimuli due to hyper-activation of the eNOS signaling cascade
- Defects in endocytosis
- the uptake of albumin by endothelial cells is drastically reduced
in these mice
- Defects in lipid homeostasis
- these mice are resistant to diet-induced obesity and have histological
abnormal adiposities with age. These mice are also hypertriglyceridemic
with a reduced capacity to clear serum lipids, a condition likely
related to the aberrant adipose function.
The phenotypes of Cav-2
null mice (Razani et al., 2002):
- Normal or slightly reduced
Cav-1 expression with no loss of caveolae - thus, these mice are extremely
useful for comparison with Cav-1 deficient mice in that they selectively
lack Cav-2
- Histologically abnormal
lungs - in fact, the lung defects in these mice are indistinguishable
from Cav-1 null mice, thereby for the first time demonstrating an
important role for Cav-2 independent of Cav-1
- Unperturbed vasoregulation
and lipid homeostasis - these observations were important in establishing
that Cav-1 and Cav-2 have distinct and non-overlapping roles in physiology
The phenotypes of Cav-3
null mice (Galbiati et al., 2001; Hagiwara et al., 2000):
- Loss of caveolae in cells
selectively expressing Cav-3 (i.e. skeletal and cardiac muscle)
- Histologically abnormal
skeletal muscle with necrotic muscle fibers and centralized nuclei
- indeed, this mild muscular dystrophy phenotype recapitulates the
pathology seen in a previously described group of patients with Limb-girdle
muscular dystrophy (type 1C) in which mutations in the CAV-3 gene
were found (Minetti et al., 1998).
- Defects in the myocyte
T-tubule network with irregularly-oriented tubules
CONCLUSIONS AND
FUTURE DIRECTIONS
As can be seen from the above description, the initial characterization
of these mice has provided a wealth of information ranging from the
predicted (e.g. involvement in endocytic processes and signaling cascades
such as eNOS) to the completely unexpected (e.g. lung hypercellularity
and defects in triglyceride rather than cholesterol homeostasis).
The study of caveolae and their marker proteins, the caveolins, has
been an exciting yet challenging endeavor. The ever-changing view of
their function in mammalian physiology is in part due to the difficulty
of working with such membrane domains and a lack of different but complementary
tools available for rigorous analyses. Caveolae and the caveolins have
thus far been implicated in endocytosis, lipid homeostasis, signal transduction,
and tumorigenesis. Now, with the availability of caveolin-deficient
mice, biochemical, cell culture, and genetic approaches can finally
be intermeshed to provide a more complete picture of caveolar function
in vivo.
REFERENCES
Anderson, H. A., Chen, Y.,
and Norkin, L. C. (1996). Bound simian virus 40 translocates to caveolin-enriched
membrane domains, and its entry is inhibited by drugs that selectively
disrupt caveolae. Mol. Biol. Cell 7, 1825-1834.
Anderson, R. G., Kamen, B.
A., Rothberg, K. G., and Lacey, S. W. (1992). Potocytosis: sequestration
and transport of small molecules by caveolae. Science 255, 410-1.
Brown, D. A., and London,
E. (1998). Functions of lipid rafts in biological membranes. Ann. Rev.
Cell Dev. Biol. 14, 111-136.
Couet, J., Sargiacomo, M.,
and Lisanti, M. P. (1997). Interaction of a receptor tyrosine kinase,
EGF-R, with caveolins. Caveolin binding negatively regulates tyrosine
and serine/threonine kinase activities. J. Biol. Chem. 272, 30429-30438.
Dietzen, D. J., Hastings,
W. R., and Lublin, D. M. (1995). Caveolin is palmitoylated on multiple
cysteine residues: Palmitoylation is not necessary for localization
of caveolin to caveolae. J. Biol. Chem. 270, 6838-6842.
Drab, M., Verkade, P., Elger,
M., Kasper, M., Lohn, M., Lauterbach, B., Menne, J., Lindschau, C.,
Mende, F., Luft, F. C., Schedl, A., Haller, H., and Kurzchalia, T. V.
(2001). Loss of Caveolae, Vascular Dysfunction, and Pulmonary Defects
in Caveolin-1 Gene-Disrupted Mice. Science 293, 2449-2452.
Engelman, J. A., Chu, C.,
Lin, A., Jo, H., Ikezu, T., Okamoto, T., Kohtz, D. S., and Lisanti,
M. P. (1998). Caveolin-mediated regulation of signaling along the p42/44
MAP kinase cascade in vivo. A role for the caveolin-scaffolding domain.
FEBS Lett. 428, 205-211.
Engelman, J. A., Lee, R.
J., Karnezis, A., Bearss, D. J., Webster, M., Siegel, P., Muller, W.
J., Windle, J. J., Pestell, R. G., and Lisanti, M. P. (1998). Reciprocal
regulation of Neu tyrosine kinase activity and caveolin-1 protein expression
in vitro and in vivo. Implications for mammary tumorigenesis. J. Biol.
Chem. 273, 20448-20455.
Feron, O., Belhhassen, L.,
Kobzik, L., Smith, T. W., Kelly, R. A., and Michel, T. (1996). Endothelial
nitric oxide synthase targeting to caveolae. Specific interactions with
caveolin isoforms in cardiac myocytes and endothelial cells. J. Biol.
Chem. 271, 22810-22814.
Fielding, C. J., Bist, A.,
and Fielding, P. E. (1999). Intracellular cholesterol transport in synchronized
human skin fibroblasts. Biochemistry 38, 2506-2513.
Fielding, C. J., and Fielding,
P. E. (1997). Intracellular cholesterol transport. J Lipid Res 38, 1503-21.
Fielding, P. E., and Fielding,
C. J. (1995). Plasma membrane caveolae mediate the efflux of cellular
free cholesterol. Biochemistry 34, 14288-14292.
Fra, A. M., Masserini, M.,
Palestini, P., Sonnino, S., and Simons, K. (1995). A photo-reactive
derivative of ganglioside GM1 specifically cross-links VIP21-caveolin
on the cell surface. FEBS Lett 375, 11-14.
Galbiati, F., Engelman, J.
A., Volonte, D., Zhang, X. L., Minetti, C., Li, M., Hou, H., Kneitz,
B., Edelmann, W., and Lisanti, M. P. (2001). Caveolin-3 null mice show
a loss of caveolae, changes in the microdomain distribution of the dystrophin-glycoprotein
complex, and T- tubule abnormalities. J Biol Chem 19, 19.
Galbiati, F., Razani, B.,
and Lisanti, M. P. (2001). Emerging themes in lipid rafts and caveolae.
Cell 106, 403-11.
Garcia-Cardena, G., Oh, P.,
Liu, J., Schnitzer, J. E., and Sessa, W. C. (1996). Targeting of nitric
oxide synthase to endothelilal cell caveolae via palmitoylation: implications
for caveolae localization. Proc. Natl. Acad. Sci., USA 93, 6448-6453.
Glenney, J. R. (1992). The
sequence of human caveolin reveals identity with VIP 21, a component
of transport vesicles. FEBS Lett. 314, 45-48.
Hagiwara, Y., Sasaoka, T.,
Araishi, K., Imamura, M., Yorifuji, H., Nonaka, I., Ozawa, E., and Kikuchi,
T. (2000). Caveolin-3 deficiency causes muscle degeneration in mice.
Hum Mol Genet 9, 3047-54.
Henley, J. R., Krueger, E.
W., Oswald, B. J., and McNiven, M. A. (1998). Dynamin-mediated internalization
of caveolae. J Cell Biol 141, 85-99.
Kerr, J., Leary, J. A., Hurst,
T., Shih, Y. C., Antalis, T. M., Friedlander, M., Crawford, E., Khoo,
S. K., Ward, B., and Chenevix-Trench, G. (1996). Allelic loss on chromosome
7q in ovarian adenocarcinomas: two critical regions and a rearrangement
of the PLANH1 locus. Oncogene 13, 1815-1818.
Koleske, A. J., Baltimore,
D., and Lisanti, M. P. (1995). Reduction of caveolin and caveolae in
oncogenically transformed cells. Proc. Natl. Acad. Sci., USA 92, 1381-1385.
Lee, S. W., Reimer, C. L.,
Oh, P., Campbell, D. B., and Schnitzer, J. E. (1998). Tumor cell growth
inhibition by caveolin re-expression in human breast cancer cells. Oncogene
16, 1391-1397.
Li, S., Couet, J., and Lisanti,
M. P. (1996). Src tyrosine kinases, G alpha subunits and H-Ras share
a common membrane-anchored scaffolding protein, Caveolin. Caveolin binding
negatively regulates the auto-activation of Src tyrosine kinases. J.
Biol. Chem. 271, 29182-29190.
Li, S., Okamoto, T., Chun,
M., Sargiacomo, M., Casanova, J. E., Hansen, S. H., Nishimoto, I., and
Lisanti, M. P. (1995). Evidence for a regulated interaction of hetero-trimeric
G proteins with caveolin. J. Biol. Chem. 270, 15693-15701.
Lisanti, M. P., Scherer,
P., Tang, Z.-L., and Sargiacomo, M. (1994). Caveolae, caveolin and caveolin-rich
membrane domains: A signalling hypothesis. Trends In Cell Biology 4,
231-235.
Lisanti, M. P., Scherer,
P. E., Vidugiriene, J., Tang, Z.-L., Hermanoski-Vosatka, A., Tu, Y.-H.,
Cook, R. F., and Sargiacomo, M. (1994). Characterization of caveolin-rich
membrane domains isolated from an endothelial-rich source: Implications
for human disease. J. Cell Biol. 126, 111-126.
Liu, P., Ying, Y., Ko, Y.-G.,
and Anderson, R. G. W. (1996). Localization of the PDGF-stimulated phosphorylation
cascade to caveolae. J. Biol. Chem. 271, 10299-10303.
Minetti, C., Sotogia, F.,
Bruno, C., Scartezzini, P., Broda, P., Bado, M., Masetti, E., Mazzocco,
P., Egeo, A., Donati, M. A., Volonte', D., Galbiati, F., Cordone, G.,
Bricarelli, F. D., Lisanti, M. P., and Zara, F. (1998). Mutations in
the caveolin-3 gene cause autosomal dominant limb-girdle muscular dystrophy.
Nature Genetics 18, 365-368.
Monier, S., Dietzen, D. J.,
Hastings, W. R., Lublin, D. M., and Kurzchalia, T. V. (1996). Oligomerization
of VIP21-caveolin in vitro is stabilized by long chain fatty acylation
or cholesterol. FEBS Lett 388, 143-149.
Monier, S., Parton, R. G.,
Vogel, F., Behlke, J., Henske, A., and Kurzchalia, T. (1995). VIP21-caveolin,
a membrane protein constituent of the caveolar coat, oligomerizes in
vivo and in vitro. Mol. Biol. Cell 6, 911-927.
Montesano, R., Roth, J.,
Robert, A., and Orci, L. (1982). Non-coated membrane invaginations are
involved in binding and internalization of cholera and tetanus toxins.
Nature (Lond.) 296, 651-653.
Murata, M., Peranen, J.,
Schreiner, R., Weiland, F., Kurzchalia, T., and Simons, K. (1995). VIP21/caveolin
is a cholesterol-binding protein. Proc. Natl. Acad. Sci., USA 92, 10339-10343.
Oh, P., McIntosh, D. P.,
and Schnitzer, J. E. (1998). Dynamin at the neck of caveolae mediates
their budding to form transport vesicles by GTP-driven fission from
the plasma membrane of endothelium. J Cell Biol 141, 101-14.
Okamoto, T., Schlegel, A.,
Scherer, P. E., and Lisanti, M. P. (1998). Caveolins, A family of scaffolding
proteins for organizing "pre-assembled signaling complexes"
at the plasma membrane. J. Biol. Chem., (Mini-review) 273, 5419-5422.
Palade, G. E. (1953). Fine
Structure of Blood Capillaries. J. Appl. Phys. 24, 1424-1436.
Parton, R. G., Joggerst,
B., and Simons, K. (1994). Regulated internalization of caveolae. J.
Cell Biol. 127, 1199-1215.
Predescu, S. A., Predescu,
D. N., and Palade, G. E. (1997). Plasmalemmal vesicles function as transcytotic
carriers for small proteins in the continuous endothelium. Am J Physiol
272, H937-49.
Razani, B., Altschuler, Y.,
Zhu, L., Pestell, R. G., Mostov, K. E., and Lisanti, M. P. (2000). Caveolin-1
Expression Is Down-Regulated in Cells Transformed by the Human Papilloma
Virus in a p53-Dependent Manner. Replacement of Caveolin-1 Expression
Suppresses HPV-Mediated Cell Transformation. Biochemistry 39, 13916-13924.
Razani, B., Combs, T. P.,
Wang, X. B., Frank, P. G., Park, D. S., Russell, R. G., Li, M., Tang,
B., Jelicks, L. A., Scherer, P. E., and Lisanti, M. P. (2002). Caveolin-1-deficient
mice are lean, resistant to diet-induced obesity, and show hypertriglyceridemia
with adipocyte abnormalities. J Biol Chem 277, 8635-8647.
Razani, B., Engelman, J.
A., Wang, X. B., Schubert, W., Zhang, X. L., Marks, C. B., Macaluso,
F., Russell, R. G., Li, M., Pestell, R. G., Di Vizio, D., Hou, H., Jr.,
Knietz, B., Lagaud, G., Christ, G. J., Edelmann, W., and Lisanti, M.
P. (2001). Caveolin-1 null mice are viable, but show evidence of hyper-
proliferative and vascular abnormalities. J Biol Chem 276, 38121-38138.
Razani, B., and Lisanti,
M. P. (2001). Caveolins and caveolae: molecular and functional relationships.
Exp. Cell. Res. 271, 36-44.
Razani, B., Wang, X. B.,
Engelman, J. A., Battista, M., Lagaud, G., Zhang, X. L., Kneitz, B.,
Hou, H., Jr., Christ, G. J., Edelmann, W., and Lisanti, M. P. (2002).
Caveolin-2-deficient mice show evidence of severe pulmonary dysfunction
without disruption of caveolae. Mol Cell Biol 22, 2329-2344.
Rothberg, K. G., Heuser,
J. E., Donzell, W. C., Ying, Y. S., Glenney, J. R., and Anderson, R.
G. (1992). Caveolin, a protein component of caveolae membrane coats.
Cell 68, 673-82.
Sargiacomo, M., Scherer,
P. E., Tang, Z.-L., Kubler, E., Song, K. S., Sanders, M. C., and Lisanti,
M. P. (1995). Oligomeric structure of caveolin: Implications for caveolae
membrane organization. Proc. Natl. Acad. Sci., USA 92, 9407-9411.
Sargiacomo, M., Sudol, M.,
Tang, Z.-L., and Lisanti, M. P. (1993). Signal transducing molecules
and GPI-linked proteins form a caveolin- rich insoluble complex in MDCK
cells. J. Cell Biol. 122, 789-807.
Scherer, P. E., Lisanti,
M. P., Baldini, G., Sargiacomo, M., Corley-Mastick, C., and Lodish,
H. F. (1994). Induction of caveolin during adipogenesis and association
of GLUT4 with caveolin-rich vesicles. J. Cell Biol. 127, 1233-1243.
Scherer, P. E., Okamoto,
T., Chun, M., Nishimoto, I., Lodish, H. F., and Lisanti, M. P. (1996).
Identification, sequence and expression of caveolin-2 defines a caveolin
gene family. Proc. Natl. Acad. Sci., USA 93, 131-135.
Schnitzer, J. E., Liu, J.,
and Oh, P. (1995). Endothelial caveolae have the molecular transport
machinery for vesicle budding, docking, and fusion including VAMP, NSF,
SNAP, annexins, and GTPases. J. Biol. Chem. 270, 14399-14404.
Schnitzer, J. E., Oh, P.,
Pinney, E., and Allard, J. (1994). Filipin-sensitive caveolae-mediated
transport in endothelium: Reduced transcytosis, scavenger endoctytosis,
and capillary permeability of select macromolecules. J. Cell Biol. 127,
1217-1232.
Shin, J. S., Gao, Z., and
Abraham, S. N. (2000). Involvement of cellular caveolae in bacterial
entry into mast cells. Science 289, 785-8.
Shridhar, V., Sun, Q. C.,
Miller, O. J., Kalemkerian, G. P., Petros, J., and Smith, D. I. (1997).
Loss of heterozygosity on the long arm of human chromosome 7 in sporadic
renal cell carcinomas. Oncogene 15, 2727-2733.
Simionescu, N., Simionescu,
M., and Palade, G. E. (1975). Permeability of muscle capillaries to
small heme-peptides: Evidence for the existence of patent transendothelial
channels. J. Cell Biol. 64, 586-607.
Simons, K., and Ikonen, E.
(2000). How cells handle cholesterol. Science 290, 1721-6.
Simons, K., and Toomre, D.
(2000). Lipid Rafts and Signal Transduction. Nat Rev Mol Cell Biol 1,
31-39.
Smart, E. J., Graf, G. A.,
McNiven, M. A., Sessa, W. C., Engelman, J. A., Scherer, P. E., Okamoto,
T., and Lisanti, M. P. (1999). Caveolins, liquid-ordered domains, and
signal transduction. Mol Cell Biol 19, 7289-304.
Smart, E. J., Ying, Y.-s.,
Donzell, W. C., and Anderson, R. G. W. (1996). A role for caveolin in
transport of cholesterol from endoplasmic reticulum to plasma membrane.
J. Biol. Chem. 271, 29427-29435.
Song, K. S., Li, S., Okamoto,
T., Quilliam, L., Sargiacomo, M., and Lisanti, M. P. (1996). Copurification
and direct interaction of Ras with caveolin, an integral membrane protein
of caveolae microdomains. Detergent free purification of caveolae membranes.
J. Biol. Chem. 271, 9690-9697.
Song, K. S., Sargiacomo,
M., Galbiati, F., Parenti , M., and Lisanti, M. P. (1997). Targeting
of a G alpha subunit (Gi1 alpha) and c-Src tyrosine kinase to caveolae
membranes: Clarifying the role of N-myristoylation. Cell. Mol. Biol.
(Noisy-Le-Grand) 43, 293-303.
Song, K. S., Tang, Z.-L.,
Li, S., and Lisanti, M. P. (1997). Mutational analysis of the properties
of caveolin-1. A novel role for the C-terminal domain in mediating homotypic
caveolin-caveolin interactions. J. Biol. Chem. 272, 4398-4403.
Tang, Z.-L., Scherer, P.
E., Okamoto, T., Song, K., Chu, C., Kohtz, D. S., Nishimoto, I., Lodish,
H. F., and Lisanti, M. P. (1996). Molecular cloning of caveolin-3, a
novel member of the caveolin gene family expressed predominantly in
muscle. J. Biol. Chem. 271, 2255-2261.
Thiele, C., Hannah, M. J.,
Fahrenholz, F., and Huttner, W. B. (2000). Cholesterol binds to synaptophysin
and is required for biogenesis of synaptic vesicles [see comments].
Nat Cell Biol 2, 42-9.
Uittenbogaard, A., Ying,
Y., and Smart, E. J. (1998). Characterization of a cytosolic heat-shock
protein-caveolin chaperone complex: involvement in cholesterol trafficking.
J. Biol. Chem 273, 6525-6532.
Yamada, E. (1955). The fine
structure of the gall bladder epithelium of the mouse. J. Biophys. Biochem.
Cytol. 1, 445-458.
Yamamoto, M., Toya, Y., Jensen,
R. A., and Ishikawa, Y. (1999). Caveolin is an inhibitor of platelet-derived
growth factor receptor signaling. Exp Cell Res 247, 380-8.
Zenklusen, J. C., Bieche,
I., Lidereau, R., and Conti, C. J. (1994). (C-A)n microsatellite repeat
D7S522 is the most commonly deleted region in human primary breast cancer.
Proc Natl Acad Sci U S A 91, 12155-12158.
Zundel, W., Swiersz, L. M.,
and Giaccia, A. (2000). Caveolin 1-mediated regulation of receptor tyrosine
kinase-associated phosphatidylinositol 3-kinase activity by ceramide.
Mol Cell Biol 20, 1507-14.
|